The SCID-hu Mouse as a Model for Human Radiation Biology
Implanting human tissues into mice lacking fully competent immune systems offers a unique opportunity to assess radiation risk in vivo.
by Seishi Kyoizumi and Mitoshi Akiyama, RERF Department of Radiobiology, Hiroshima
This article was originally published in RERF Update 4(2):3-4, 1992.
The radiobiological study of humans has been hampered by a lack of suitable in vivo experimental models. Of course, acute and chronic radiation effects in humans have been documented in the studies of atomic bomb (A-bomb) survivors and patients irradiated either by therapeutic intent or by accident. However, the information gained from these studies has been limited by the difficulties in estimating precise radiation doses and in obtaining biological samples for directly analyzing the processes of radiation-induced pathogenesis. In vitro, it has been possible to deduce simple survival data for human clonogenic cells, such as skin fibroblasts, T lymphocytes, and bone marrow CFU-C(colony forming unit-culture) under defined conditions. However, it is not clear whether such in vitro conditions adequately reflect the complex microenvironmental conditions that exist in vivo. Given these limitations, meaningful risk estimates are difficult to achieve. With these issues in mind, we propose that the severe combined immunodeficient mouse-human (SCID-hu) chimera can be used as an in vivo experimental model for human radiation biology.
The SCID-hu mouse model
In 1988, J. M. McCune and colleagues at SyStemix Inc, Palo Alto, Calif, succeeded in implanting functional human fetal hematolymphoid organs including thymus and lymph node tissues into the severe combined immunodeficient (SCID) mouse (JM McCune et al, Science 241:1632-9, 1988). Recently, we reported successful implantation of functional human fetal bone marrow into the mouse (S Kyoizumi et al, Blood 79:1704-11, 1992). Other human tissues, such as skin, lung, and colon, were also found to be transplantable and morphologically normal in SCID mice (personal communication with R Namikawa et al, SyStemix Inc, Palo Alto, Calif). Such a chimeric mouse (ie, the SCID-hu mouse) can be experimentally irradiated with any type and dose rate of ionizing radiation to analyze in vivo effects of radiation on human tissues (Figure 1).
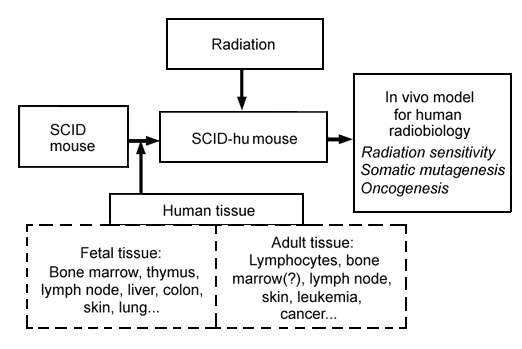
Figure 1. Applications of the SCID-hu mouse system to studies of human radiation biology.
As a result, it may now be feasible to conduct analyses of in vivo radiation sensitivity of human tissues, as well as studies in vivo of radiation-induced somatic mutagenesis, such as specific gene mutations and chromosome aberrations. We also might be able to study transformation or oncogenesis in these irradiated human tissues followed by appropriate promotions. Using this experimental system, we may be able to answer many questions about the mechanisms for radiation-induced carcinogenesis (JE Trosko, RERF Update4(1):3-5, 1992). Thus, it is expected that this SCID-hu model may provide complementary and supportive data for the studies of A-bomb survivors and accidentally exposed people. Since the conclusions from the study using this model would be drawn directly from observations of human tissues in vivo, it is likely that they will contribute more relevant and meaningful insights to human radiation biology than those obtained in vitro or from other nonhuman animal models.
Here we will summarize briefly the effects of radiation on human bone marrow engrafted into SCID mice. We will also discuss a possible application of this bone marrow model to the study of somatic mutations in hematopoietic stem cells of A-bomb survivors.
Effect of radiation on human hematopoiesis in the SCID-hu mouse
The SCID-hu bone marrow model was created by surgically implanting human fetal bone fragments (between the 18th and 22nd gestational weeks) into SCID mice (S Kyoizumi et al, Blood 79:1704-11, 1992). The advantage of implanting bone fragments is that not only hematopoietic stem cells but also the microenvironment required for hematopoiesis can be transferred to the mice. The implantation process was associated with an immediate decline in hematopoietic activity in the bone grafts, followed by a recovery. Histologic examination at 2-3 weeks showed necrotic changes in the marrow and no clear foci of hematopoietic cells were observed. After 6-8 weeks, most of the grafts looked morphologically similar to normal human bone marrow: a high degree of cellularity was associated with human lymphopoiesis, myelopoiesis, erythropoiesis, and megakaryocytopoiesis. More than 90% of the bone grafts demonstrated and maintained such signs of hematopoiesis for as long as 20 weeks. Human progenitor cell activities for multiple lineages, including myeloid progenitors (colony-forming unit-granulocyte macrophage, CFU-GM) and erythroid progenitors (burst-forming unit-erythroid, BFU-E), also were demonstrated in the methylcellulose colony assay. Flow cytometric analysis demonstrated that the cells recovered from CFU-GM and BFU-E colonies expressed human lineage-specific markers, CD15 and glycophorin A, respectively. Constant levels of progenitor activity within the range normally found in human bone marrow were maintained in the grafts for longer than 20 weeks. These findings demonstrate that a suitable microenvironment for maintaining human stem cells and for inducing their differentiation can be successfully introduced into SCID mice.
Using this SCID-hu model, it is possible to derive quantitative data about radiotoxic effects on human hematopoiesis in vivo (S Kyoizumi et al, Radiat Res 137:76-83, 1994). After whole-body X-irradiation, human fetal bone marrow implanted into SCID-hu mice showed a typical radiation sensitivity for mammalian hematopoietic progenitor cells. The survival curves for CFU-GM and BFU-E were found to have no shoulders, and the D0 value was 1.0 Gy for CFU-GM and 0.7 Gy for BFU-E. This D0 value of CFU-GM is similar to that found for human bone marrow CFU-GM irradiated in vitro, as well as for mouse CFU-S (colony-forming unit-spleen) and CFU-GM irradiated in vivo (JH Hendry, Int J Radiat Biol47:3-16, 1985). Greater sensitivity of erythroid progenitors to radiation also has been reported for mouse and dog bone marrow. These data suggest that the radiobiological characteristics of human hematopoietic progenitor cells can be maintained in the bone grafts. Also, this X-ray-induced acute hematotoxicity was significantly reduced when the well-known radioprotective agent WR-2721 (a free radical scavenger) was administered before irradiation. The degree of the dose-reduction effect of WR-2721 was very similar to that reported for normal mouse bone marrow. Furthermore, after low-dose irradiation (less than 1.5 Gy of X-irradiation), hematopoietic functions in the bone marrow graft could recover to the normal level. Previous studies in human clinical trials have demonstrated that human granulocyte-colony-stimulating factor (G-CSF) and granulocyte macrophage colony-stimulating factor (GM-CSF) can promote hematopoietic recovery after myelosuppression caused by radiation exposure (RP Gale and A Butturini, Exp Hematol 18:958-64, 1990). We demonstrated that recovery of human CFU-GM activity in SCID-hu mice was accelerated by the administration of human G-CSF, and neutrophil populations remarkably increased within the bone marrow grafts. Thus, human G-CSF augmented myelopoiesis by stimulating myeloid progenitor cells that survived radiation exposure.
These studies have demonstrated that the SCID-hu model may prove suitable for risk analysis of human radiation exposure, as well as for developing new modalities for preventing and treating radiotoxic damage to the human hematopoietic system.
Applications to somatic mutation studies of A-bomb survivors
The SCID-hu bone implant model might be used to analyze other features of human bone-marrow radiobiology, especially radiation-induced mutations in hematopoietic progenitor cells of A-bomb survivors (Figure 2). An elevated somatic mutation frequency in erythrocyte glycophorin A genes was detected in A-bomb survivors more than 45 years after radiation exposure (R Langlois et al, Science 236:445-8, 1987; S Kyoizumi et al, Cancer Res 49:581-8, 1989; M Akiyama et al, J Radiat Res 32(Suppl):278-82, 1991). Also, common HPRT gene mutations and chromosome aberrations were found among various T- and B-cell clones isolated from the same survivors (M Hakoda et al, J Exp Med169:1265-76, 1989; Y Kusunoki et al, Blood 86:2106-12, 1995). These observations suggest that radiation-induced mutations were recorded in bone marrow stem cells and that mutant stem cells continuously supplied mutant mature blood cells in A-bomb survivors. Because this conclusion was based on observations from analyses of mature blood cells, direct demonstration of such mutations in hematopoietic progenitor or stem cells is being sought eagerly.
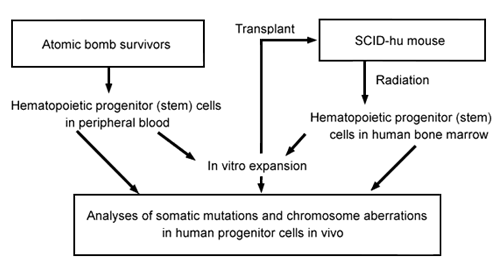
Figure 2. Strategies of studies on radiation-induced somatic mutations in hematopoietic progenitor cells.
One approach is to analyze somatic mutations and chromosome aberrations induced in progenitor cells isolated from SCID-hu mice after radiation exposure (Figure 2). Another is to isolate hematopoietic progenitor cells directly from the peripheral blood of A-bomb survivors. Recent advances in stem-cell technology make it possible to purify hematopoietic progenitor cells and to expand them in culture containing various hematopoietic growth factors including stem cell growth factor (ON Witte, Cell 63:5-6, 1990). Using this technique in studies of A-bomb survivors, we might be able to demonstrate and measure directly somatic mutations in hematopoietic progenitor cells circulating in the peripheral blood. Furthermore, these mutant progenitor cells might be transferable to SCID mice, where they might differentiate to mature blood cells in the mice after administration of various human hematopoietic growth factors. We hope these two approaches will systematically demonstrate the dynamics of generation and differentiation of mutant stem cells after radiation exposure.